Using light, we are able to image the living brain in a number of different ways to be able to visualize both the neuronal and hemodynamic behavior of the brain at ensemble and cellular levels. Optical neuroimaging is a major focus of our lab, and in particular, we are seeking to use our imaging systems to better understand neurovascular coupling and cerebral metabolism.
A local increase in cortical blood flow accompanies almost all neuronal responses to stimulus in the brain. This hemodynamic response is the basis of functional magnetic resonance imaging (fMRI). However, surprisingly little is understood about the interrelation between blood flow and the neuronal activity that underlies it. It would be of significant value to be able to interpret clinical fMRI data in terms of neuronal function. Yet more importantly, normal functioning of the brain seems to depend critically on the integrity of neurovascular coupling, so understanding the mechanistic basis of this coupling could yield therapeutic targets for a range of pathologies including Alzheimer's and age related neurodegeneration.
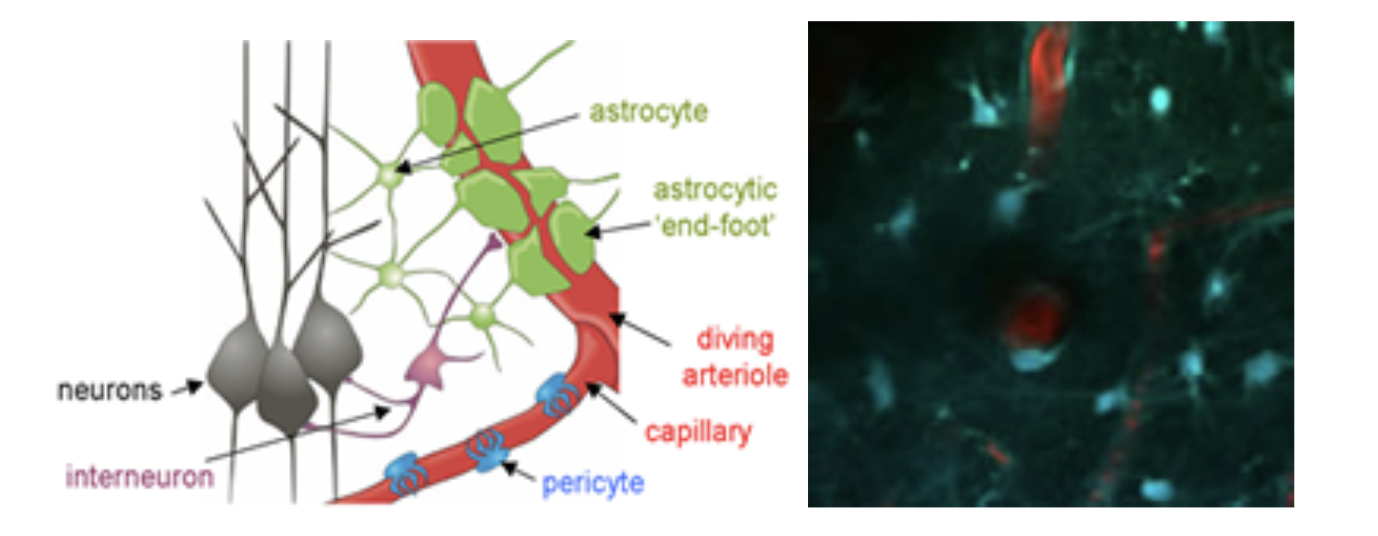
Left: Elements of the neurovascular unit. Astrocytes, interneurons, pericytes and the blood vessels themselves are all suspected to play a role in orchestrating the hemodynamic response to stimulus. *Adapted from: Gordon, Mulligan & MacVicar, Glia ,2007. Right: in-vivo two-photon image of the interactions between blood vessels and astrocytes.
Studying neurovascular coupling is complex owing to the need for both neuronal interconnectivity and vascular networks to be intact. In-vivo imaging allows the complete neurovascular system to be observed, perturbed and directly linked to clinical measures such as fMRI. However in-vivo imaging presents significant challenges in terms of experimental paradigm and instrument design. Advanced imaging techniques are required to capture the behavior of the brain in real time, measuring both vascular and neuronal parameters in parallel, and probing beneath the surface of the cortex to resolve 3D and layer-specific interactions. We utilize all of our imaging tools described here to investigate neurovascular coupling in the living brain.
Hillman EMC, "Out for Blood", Scientific American MIND, July / Aug (2014).
Hillman EMC, "Coupling Mechanism and Significance of the BOLD Signal: A Status Report", Annual Reviews, Neuroscience, 37, 161-181 (2014)
Our high-resolution, high-speed multispectral optical imaging techniques give us a unique view of the brain. While the fMRI response is typically observed at frame rates of less than 1Hz, and with voxel sizes exceeding 2-3mm, we can resolve the behaviors of individual vessels, informing the interpretation of the ensemble signal detected by fMRI. Multi-spectral optical intrinsic signal imaging (MS-OISI) can also distinguish between changes in oxy-, deoxy- and total hemoglobin (HbO, HbR and HbT). The fMRI blood oxygen level dependent (BOLD) signal predominantly shows only changes in HbR, which can make it difficult to disambiguate changes in total hemoglobin from changes in oxygenation.
Figure 2 below shows a collection of our work characterizing the hemodynamic response in the rat somatosensory cortex to fore- and hind-paw stimulation. The typical hemodynamic response can be seen to consist of a rapid increase in HbT and HbO with a concomitant decrease in HbR (the latter of which constitutes a ‘positive BOLD’ response. This response corresponds to a local increase in blood flow and a resulting increase in the amount of oxygenated blood present in the region (evidence of marked increases in oxygen consumption are most often masked by this larger hyperemic response). Examining how this response is generates, we can look at the dynamic changes in diameter of the pial vessels that feed the deeper capillary beds of the cortex. We reported in both Hillman et al, 2007 and Bouchard et al, 2009 that rapid dilation of the pial arterioles can be clearly observed, whereas little or no dilation (passive or active) of pial veins occurs with standard (4 second, 3Hz) stimulation. This result was confirmed using LOT, high-resolution MS-OISI and dynamic in-vivo two-photon microscopy. The latter two techniques also allowed us to quantitatively evaluate the speed of blood flow in pial veins from the movement of red blood cells, and in both cases, significant flow increases were seen, consistent with a lack of significant dilation (and consistent in magnitude with the expected flow increases from arteriolar dilation). This result has important implications for modeling of fMRI data, which had previously assumed that the majority of HbT changes occurred in the venous compartment. Instead, our results suggest that the capillary beds are highly compliant.
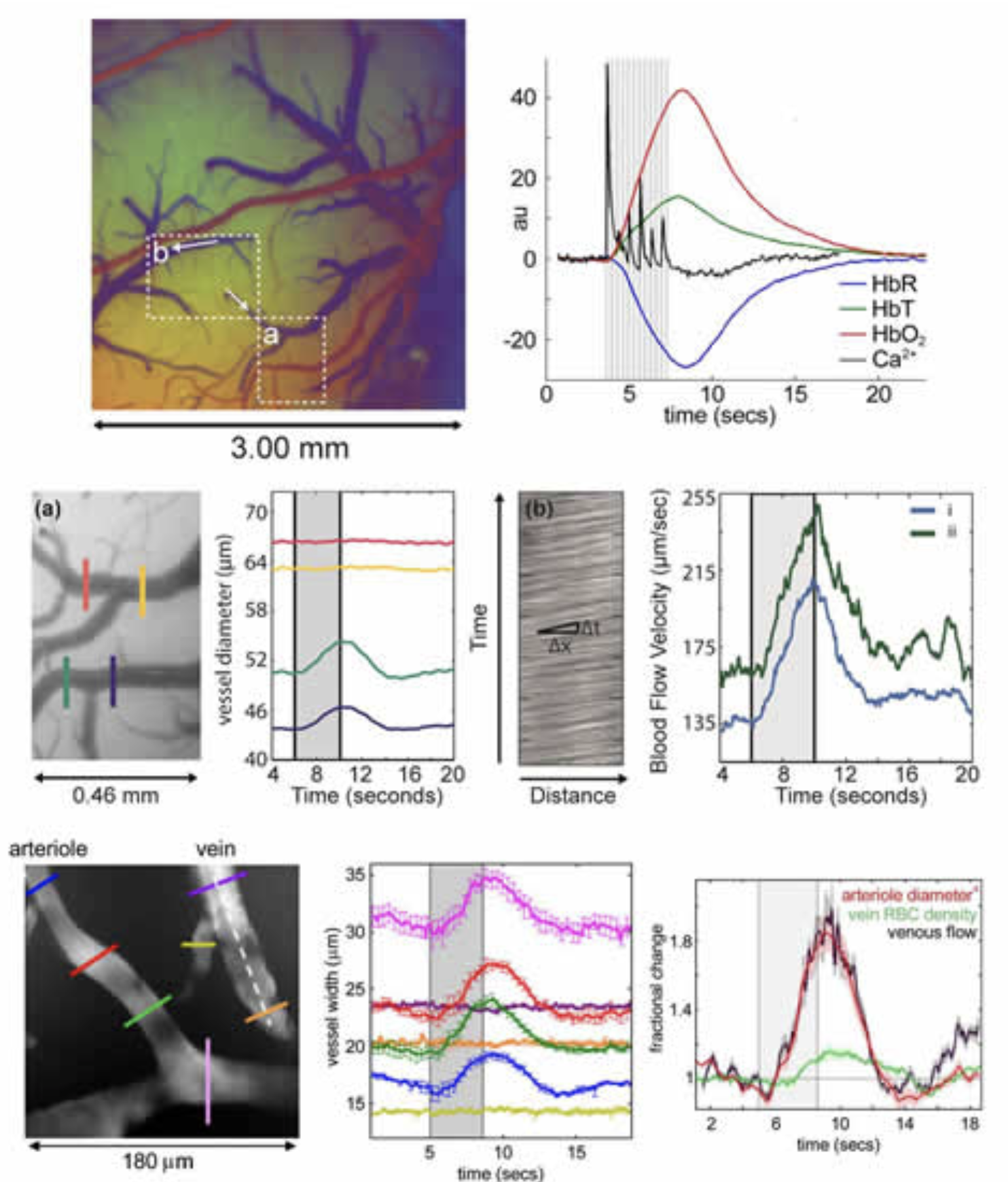
Vascular compartment-resolved dynamics. Top left: image of the exposed cortex color-coded according to baseline oxygenation such that arterioles appear red and veins appear blue. Top right: A standard cortical hemodynamic response to 4 second somatosensory stimulation overlaid with signal from calcium sensitive dye imaging representing the neuronal response in the same region. 2nd row: zoomed region from inset (a) in the top left image and the dilation dynamics of two vessels during stimulation. The vein (red, yellow) shows no dilation, while the arteriole (green, blue) does. Right: the motion of blood in the veins in inset region (b) allows quantitative calculation of blood flow changes. Both branches show marked increases in the speed of flow. 3rd row: Dynamic in-vivo two-photon measurements of pial vessels (after IV FITC dextran injection) showing the same marked dilation of arterioles with no dilation of venules – but with increases in venous speed of flow. Plot to right shows that percentage increase in flow from arteriolar dilation (diameter to the 4th power per Bernoulli) matches the percentage increase in venous flow. Figure modified from Bouchard et al, 2009 and Hillman et al, 2007.
Extending the above studies, we then sought to answer whether arteriolar dilation precedes or follows changes in the capillary bed, and whether we could resolve whether arteriolar dilation propagates and if so, in which direction (retrograde or anteriograde). Using statistical methods to determine the very earliest time of ‘response onset’ across the responding region imaged using MS-OISI we found that HbT changes in the parenchyma originating from the capillary bed precede dilation of pial arterioles by a few hundred milliseconds. We observed high speed (2-4 mm/s) retrograde propagation of vasodilation in the pial arteries feeding the responding region, and found that this dilation spread independent of the direction of blood flow in the vessels. Dilations over 1mm away were seen within 400 milliseconds of stimulus onset. We also evaluated the dynamics of the return to baseline, and found markedly different spatiotemporal characteristics, suggesting the interplay of two processes; one dilatory and one driving constriction over different spatiotemporal scales. Chen et al, 2011. These results provide benchmark characteristics of the hemodynamic response to give a better framework in which to evaluate different candidate cellular mechanisms for neurovascular control.
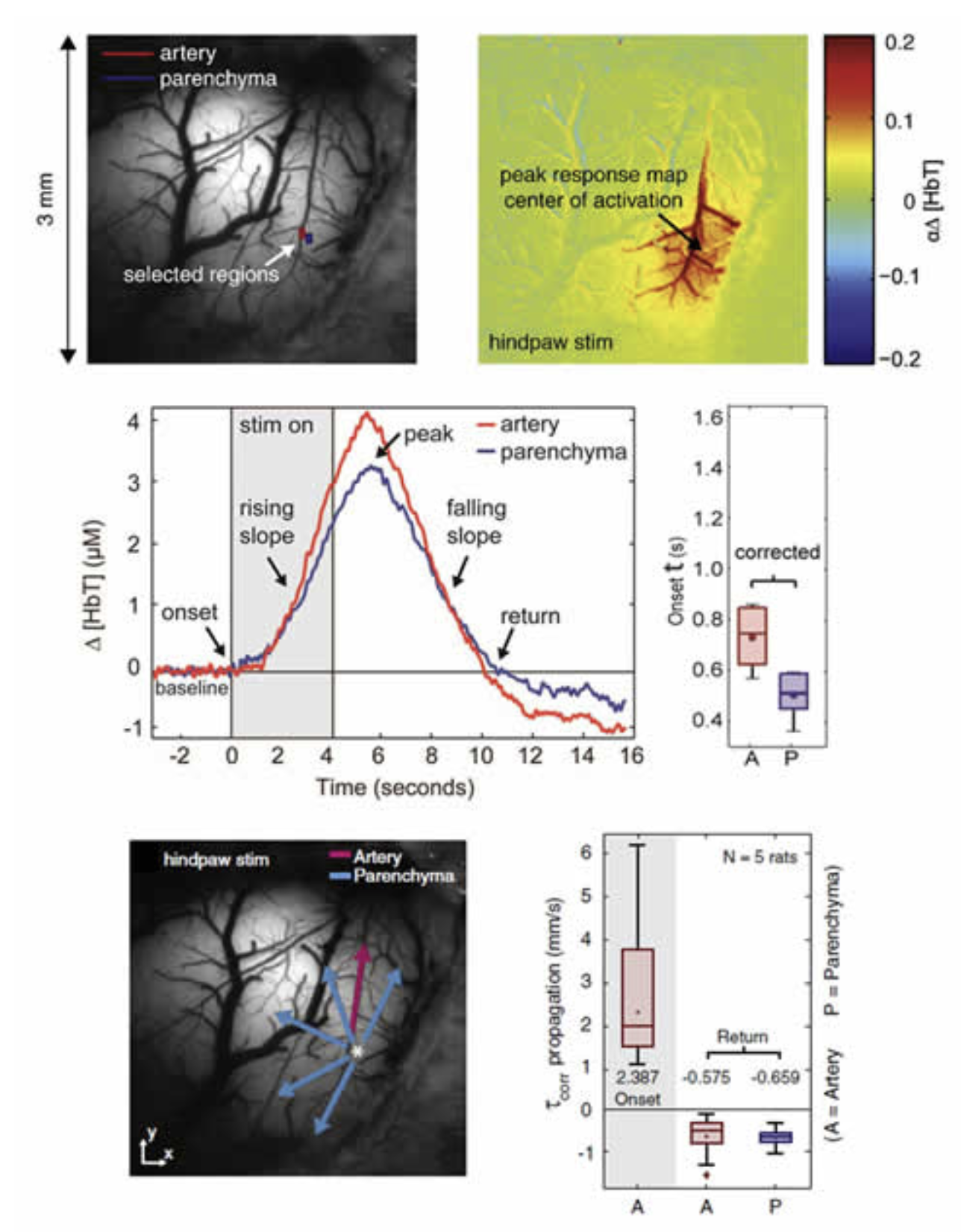
High-speed imaging of the hemodynamic response. Top left: image of the exposed somatosensory cortex under 530nm illumination. Right: Map of the change in HbT at the peak of the hemodynamic response. Increases in HbT can be seen in the parenchyma (reflecting increases in capillary perfusion) and in key branches of the arterioles feeding the active region. 2nd row: time-courses taken from the regions indicated in the image top-left showing the dynamics of the response onset and return to baseline. Plot to right shows a measure of the onset time (time to cross a statistically-defined threshold) for pial arteries (A) and the parenchyma (P). 3rd row: Mapping the directional speed of propagation of HbT increases revealed arteriolar dilation spreading away from the responding region, irrespective of blood flow direction. The periphery returned to baseline first, followed by the central region, at much slower speeds than the onset. Figure modified from Chen et al, 2011.
Hillman EMC, Devor A, Bouchard M. B, Dunn A. K, Krauss GW, Skoch J, Bacskai J, Dale A. M, Boas D. A. “Depth-resolved Optical Imaging and Microscopy of Vascular Compartment Dynamics During Somatosensory Stimulation”, NeuroImage, 35(1): 89-104 (2007)
Chen BR, Bouchard MB, McCaslin AFH, Burgess SA, Hillman EMC, "High-speed vascular dynamics of the hemodynamic response", Neuroimage, 54 (2), 1021-1030 (2011).
Astrocytes possess many properties that make them attractive candidates for involvement in neurovascular coupling. They can sense the presence of local glutamate release via their metabotropic glutamate receptors, and can release a range of vasoactive products including prostaglandins and potassium ions. They are also well documented to be present around vessels in the brain. Astrocytes have been shown to exhibit transient increases in intracellular calcium during stimulation, and photolysis-induced changes in intracellular calcium have been shown to correlate with dilation of local diving arterioles (See Takano et al, 2005 and Wang et al, 2006). However, the morphology of astrocyte connectivity with the cortical vasculature can be difficult to evaluate using ex-vivo histology, where blood vessels are not perfused and the pial surface is rarely intact or considered. We therefore undertook to survey the relative interactions between astrocytes and their endfeet and the different vascular compartments of the in-vivo cortical vasculature. Astrocytes were labeled with sulforhodamine 101 (SR101) and the vasculature was loaded with FITC dextran. Extensive 3D analysis revealed that astrocytes enstheath all sub-pial vessels; diving arterioles, capillaries and ascending veins. There is little difference between this sheathing except that more astrocyte cell bodies, on average, are present on diving arterioles. The extensiveness and continuity of this sheathing suggests that it could act as a conduit for signal transduction. Sheathing of diving veins was seen to extend from the glia limitans superficialis with no SR101 staining seen on pial veins. On diving arterioles, SR101 staining was observed, but was seen as an inner sheath, with a second external sheath of astrocyte processes joining arterioles as they dive through the glia limitans.
Immunohistochemistry confirmed that the inner staining of the pial and diving arterioles corresponds to uptake of SR101 into arterial endothelial cells, and does not indicate the presence of astrocytes. This endothelial uptake was not seen in veins. Figure 4 below shows further results from this study where the depth-dependent density of astrocyte cell bodies and microvasculature were compared and found to exhibit little correlation. However, despite the substantial variations in relative density, astrocytes were found to be located at relatively consistent distances from one another as a function of depth (averaging ~ 6 microns). See McCaslin et al, 2011.
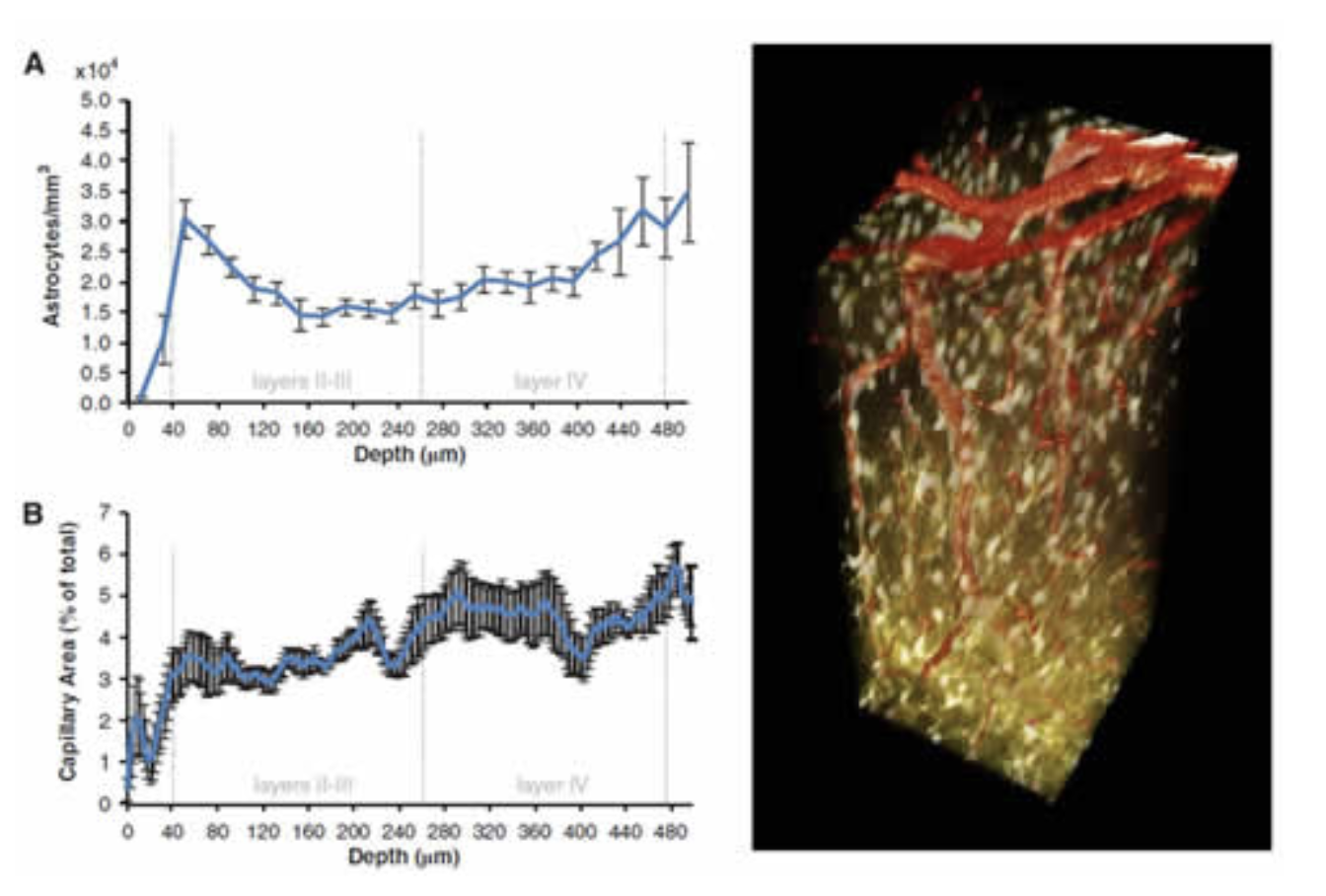
In-vivo imaging of astrocyte morphology. Right; 3D rendered volume showing in-vivo astrocytes labeled with SR101 (gold) and vasculature carrying FITC dextran (red). Left top; density of astrocyte cell bodies as a function of cortical depth. Left bottom: Microvascular density as a function of cortical depth. Figure modified from McCaslin et al, 2011.
We interpret these results as evidence that astrocytes are indeed intimately related to the vasculature, but that they do not exhibit preferential relationships with diving arterioles. We also note the lack of connectivity between the astrocyte network and the pial arterioles. Comparing this morphology to our dynamic imaging results, astrocytes seem unlikely to be the primary mediators of long-distance, rapid dilation of pial arteriole branches.
McCaslin AFH, Chen BR, Radosevich AJ, Cauli B, Hillman EMC, "In-vivo 3D morphology of astrocyte-vasculature interactions in the somatosensory cortex: implications for neurovascular coupling", J Cereb
Blood Flow Metab, 31, 795-806 (2011).
In ongoing studies, we are using dynamic in-vivo two-photon microscopy of calcium sensitive dyes to map the neuronal and astrocytic responses to somatosensory stimulation. By establishing accurate spatiotemporal assessment of their responsivities, we hope to clarify the role of astrocytes in neurovascular coupling. An example of this approach is shown below in Figure 5 which shows wide-field MS-OISIimaging of the exposed cortex after multiple intracortical injections of Oregon Green 499 BAPTA-1 AM calcium sensitive dye. After observing the locations of both the hemodynamic and neuronal responses, we can use two-photon microscopy in the same animal to zoom in an evaluate the responses of individual cells at different depths.
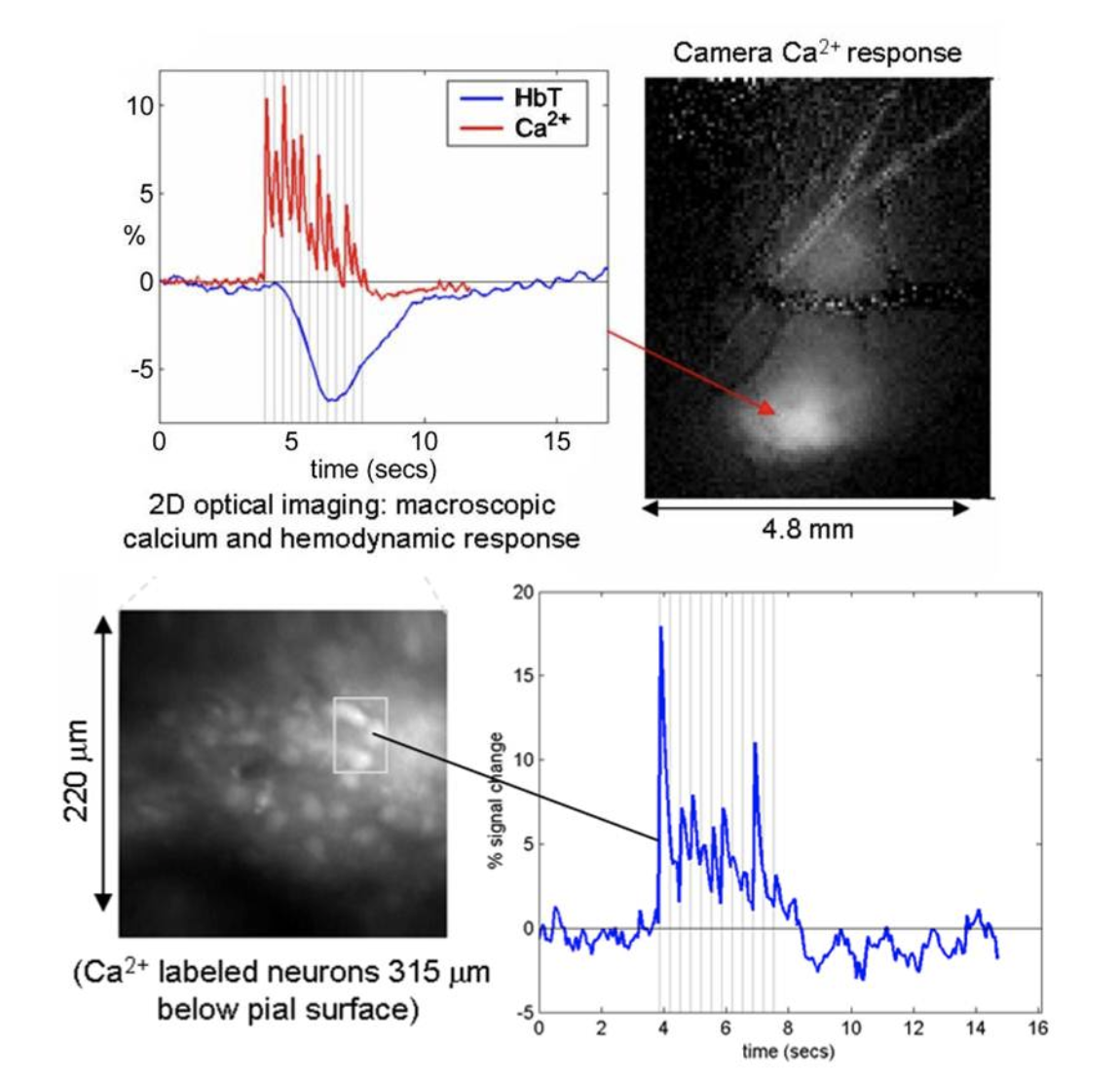
Left: camera-based imaging of the ensemble response to 3Hz forepaw stimulus in the exposed somatosensory cortex. Right: high-speed two-photon imaging of the same response in the region shown. See Hillman 2007 for more details.
Bouchard MB*, Chen BR*, Burgess SA, Hillman EMC, "Ultra-fast multispectral optical imaging of cortical oxygenation, blood flow and intracellular calcium dynamics", Optics Express, 17 (18), 15670-15678, (2009).* Equal contribution first authors.
In collaboration with Aniruddha Das and Yevgeniy Sirotin, we completed a study using MS-OISI data acquired on the exposed visual cortex of awake behaving primates to demonstrate an important confound in conventional optical intrinsic signal imaging (OISI). In conventional OISI, many investigators use only a single wavelength of light to image the cortex, typically between 610-630nm. It is expected that this wavelength is predominantly sensitive to changes in deoxy-hemoglobin (HbR) since only oxy-hemoglobin (HbO) only contributes a small amount to absorption at these wavelengths (see Figure 6 below). However, data acquired at these ‘oximetric’ wavelengths typically has a biphasic shape, initially showing a decrease in detected light, followed by an increase. Simple interpretation of this pattern would suggest that HbR levels are initially increasing (termed the ‘initial dip’) and then subsequently decrease – and this pattern has been widely interpreted as measuring an initial, local increase in oxygen consumption (converting HbO to HbR) followed by a wash-in effect due to active local increases in blood flow and therefore HbT.
Our work demonstrated that the initial decrease in signal at these oximetric wavelengths is in fact driven by a large local increase in HbT (consisting of both HbO and HbR) rather than a selective increase in HbR in the absence of an increase in HbT. We demonstrated that by converting raw MS-OISI data into changes in concentration of HbO, HbR and HbT (via careful Monte Carlo modeling that accounted for a variety of different assumptions about scattering and its wavelength dependence) that data that appeared to show a dip corresponds well to a response in which no initial increase in HbR is observed, only a rapid HbT increase. This can be readily confirmed by imaging at 530nm, an isobestic point sensitive only to HbT, which clearly shows a rapid HbT increase. Interestingly, one of the attractive features of the ‘initial dip’ is that it is generally spatially constrained to a fairly small area of the capillary bed, appearing to be a localized, more neuronally-coupled signal than the larger ‘rebound’ of increased blood flow involving the pial arteries and veins. However, this observation is consistent with our work described above which suggests that the first part of the hemodynamic response is a very rapid, localized increase in capillary HbT.
Note: our results don’t rule out the possibility that a genuine initial dip (increase in HbR) could occur in some situations where oxygen consumption precedes or exceeds increases in local perfusion. However, it does demonstrate that measurements made at 605-630 nm are likely to show a biphasic signal that could be interpreted as an initial dip and rebound even in cases where no HbR decrease is actually occurring and changes can be attributed to rapid local increases in HbT. For details see Sirotin et al, 2009.
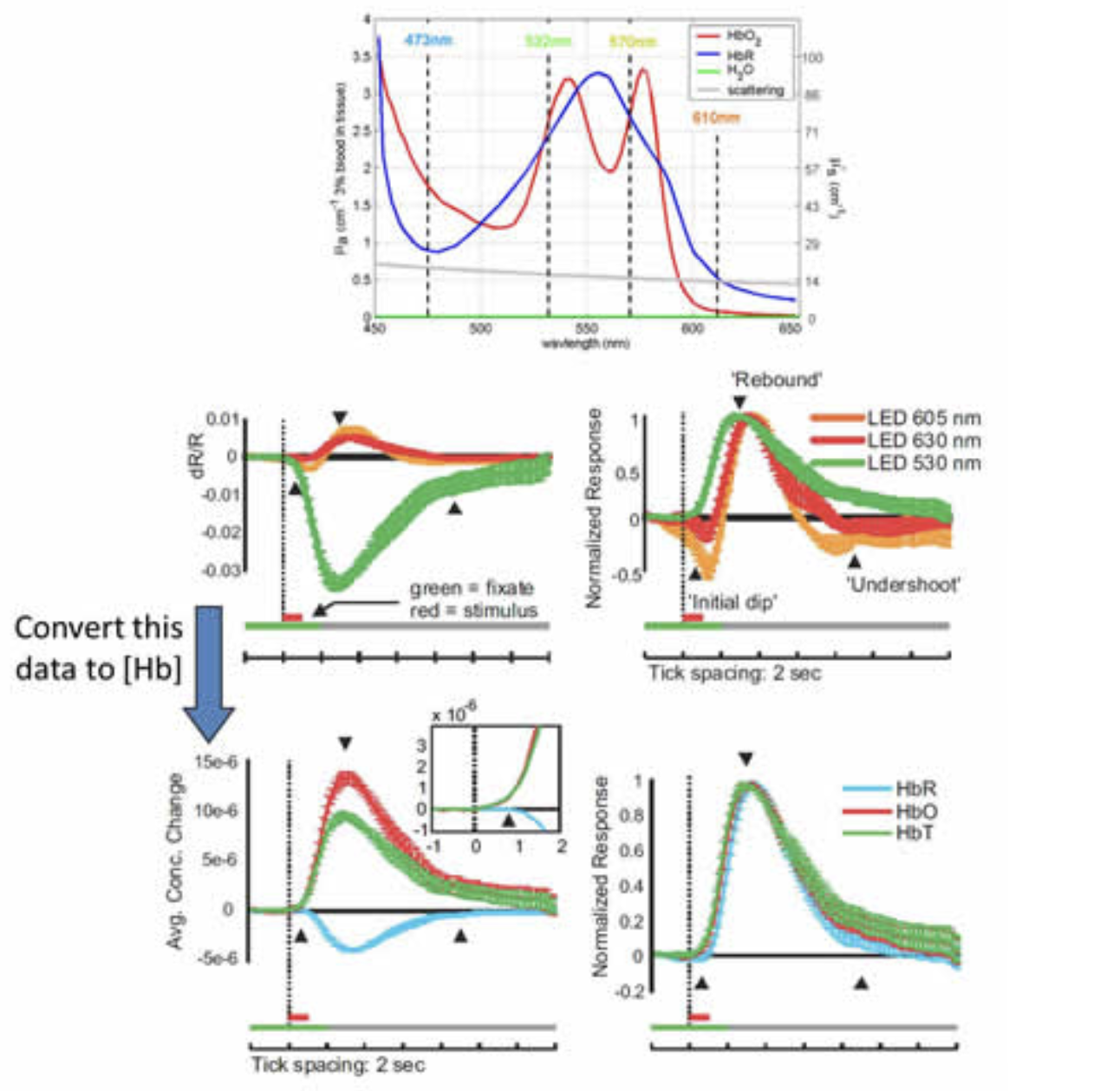
Reinterpreting the ‘initial dip’ at 605-630nm. Top: Absorption spectrum of oxy- and deoxy-hemoglobin. Middle row: left: relative signal changes in visual cortex observed during and after a short visual stimulation measured at three different wavelengths. Right: normalized data shows large undershoot in signals from wavelengths > 600 nm. Bottom row: When this same data is converted to changes in concentration of hemoglobin using modified Beer-Lambert calculations with wavelength dependent pathlength calculated from Monte Carlo modeling, the result shows a response with a rapid increase in HbT and HbO and a slightly delayed decrease in HbR, but no marked increase in HbR. Right: Normalized data showing rapid HbT increase. Modified from Sirotin et al, 2009 and Hillman, 2007.
Sirotin YB, Hillman EMC, Bordier C, Das A. "Spatiotemporal precision and hemodynamic mechanism of optical point-spreads in alert primates", PNAS 106, 18390-18395, (2009).
We recently demonstrated that the vascular endothelium plays an important role in neurovascular coupling in the brain (Chen et al, 2014, Hillman, 2014). We propose this model based on our many observations of high-speed propagated dilation of brain arterioles.
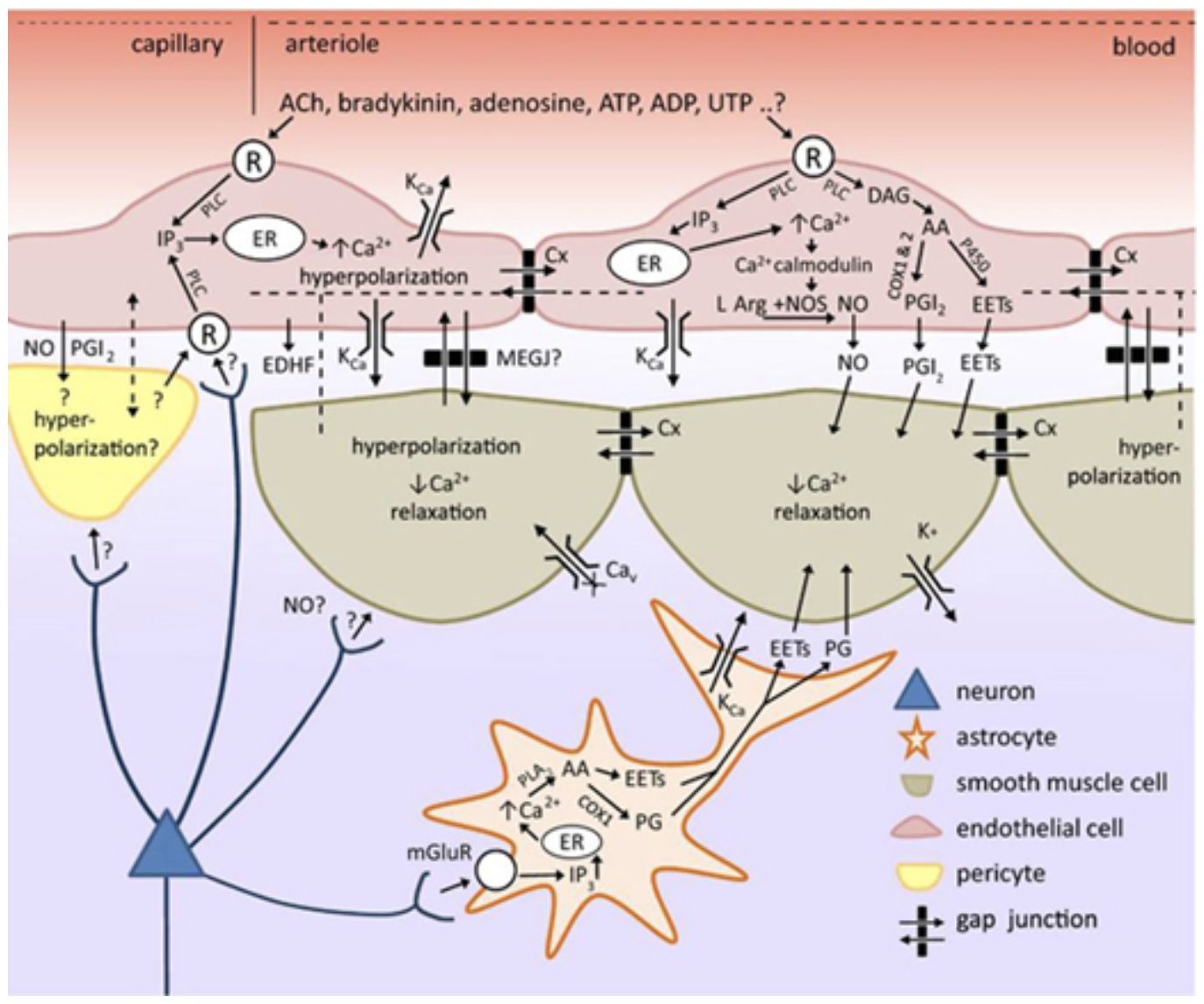
Adding the vascular endothelium to our picture of neurovascular coupling. We note that endothelial cells can feasibly use the same pathways to alter vascular smooth muscle tone as astrocytes. Endothelial cells can also propagated vasodilation long distances along a vessel, utilizing at least two different processes: high-speed endothelial hyperpolarization and a slower process accompanied by a calclium wave that elicits dilation via prostanoids, NO and / or EETs. This intravascular pathway for propagating vasodilation makes it feasible that neurovascular coupling is initiated at the level of capillaries, and propagates rapidly to upstream penetrating and pial arterioles. Brain endothelial cells express a wide range of receptors that have yet to be explored as possible components in neurovascular coupling. See Chen et al 2014 and for full legend.
To demonstrate the role of the endothelium, we developed an in-vivo light-dye technque to cause spatially selective damage to the endothelium of pial arteries, leaving the rest of the vessel intact.
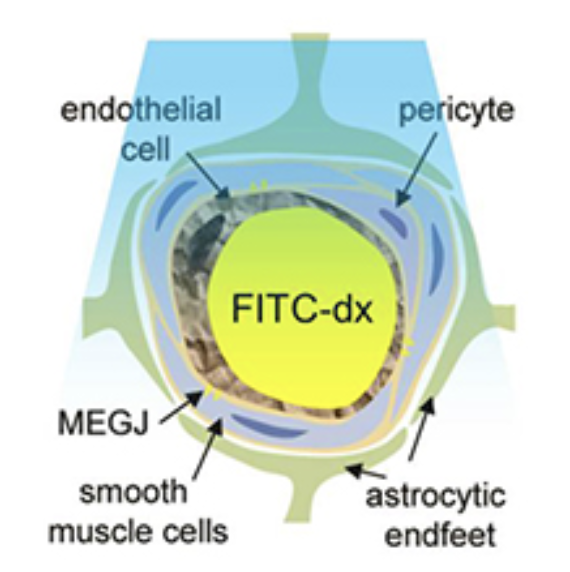
FITC within the vessel releases oxygen free radicals upon blue light illumination. These directly (and selectively) damage the vessel's inner endothelial layer, while leaving the rest of the vessel intact. The use of light means that different patterns of endothelial disruption can be achieved in the intact, in-vivo brain.
In Chen et al 2014, we showed that pial arteries that previously dilated in response to somatosensory stimulation did not dilate beyond the point of light-dye damage (shown below). Wide-field light-dye prevented any dilation of pial arteries, which led to a significant reduction in functional hyperemia (as measured by HbT).
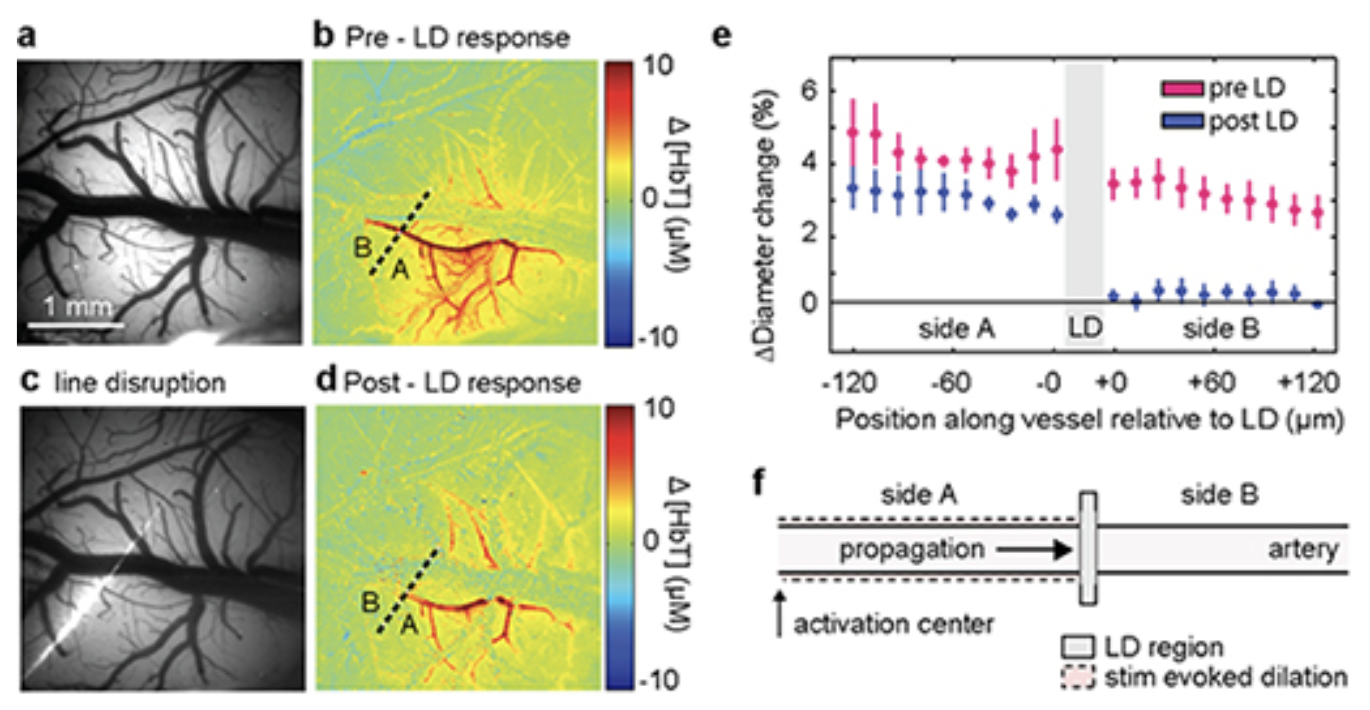
A fine line of laser light disrupts a small section of arterial endothelium in the somatosensory cortex. Before disruption, the targeted pial arteriole dilated rapidly following somatosensory stimulation. After light-line damage, dilation abruptly halts at the point of endothelial damage. See Chen et al 2014 and for full legend.
Control experiments confirmed that the damaged part of the vessel still dilated in response to sodium nitroprusside, confirming that the vessel's smooth muscle was still intact. Acetylcholine evoked dilation of all parts of the pial arterioles except the regions directly illuminated during light-dye (including the 'distal' portion of the arteriole in light-line experiments that had failed to dilate in response to somatosensory stimulation).
We conclude that the vascular endothelium forms an essential conduit for conduction of vasodilatory signals along the arteries and arterioles feeding active neurons. This finding could resolve many of the discrepancies in earlier models of neurovascular coupling.
Chen BR, Kozberg MG, Bouchard MB, Shaik MA, Hillman EMC, "A critical role for the vascular endothelium in functional neurovascular coupling in the brain", JAHA, 3: e000787, (2014).
Hillman EMC, "Coupling Mechanism and Significance of the BOLD Signal: A Status Report", Annual Reviews, Neuroscience, 37, 161-181 (2014).
In a recent collaboration with Drs Guy McKhann, Jeffrey Bruce and Peter Canoll at Columbia, we have developed an MS-OISI system capable of acquiring high-resolution, high-speed data on the exposed cortex of humans during neurosurgery. This data, combined with intrasurgical electrocorticography is giving us unique insights into resting state and stimulus-evoked fluctuations in the anesthetized and awake human brain.
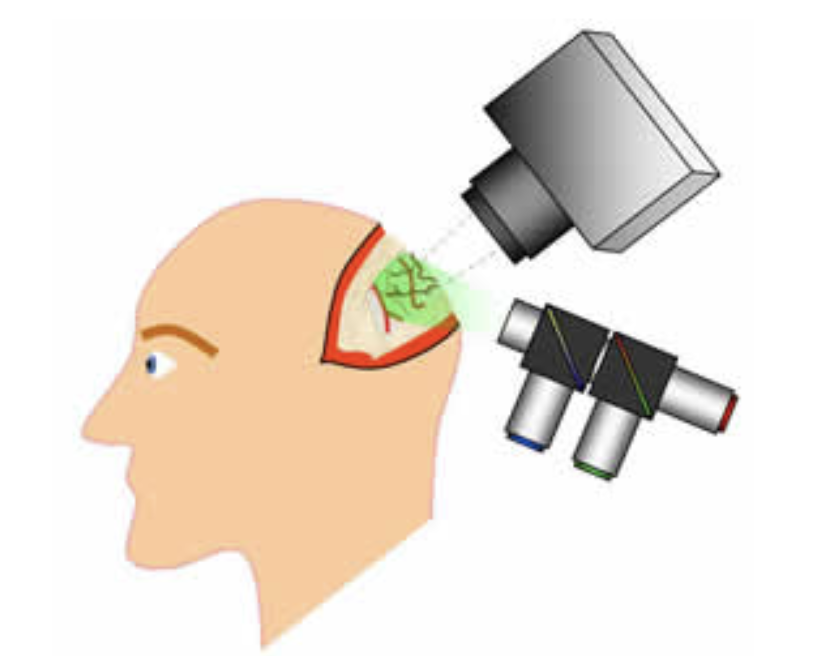
Schematic of exposed-cortex multi-spectral optical intrinsic signal imaging during human neurosurgery
Rayshubskiy A, Wojtasiewicz TJ, Mikell CB, Bouchard MB, Timerman D, Youngerman BE, McGovern RA, Otten ML, Canoll, PD, McKhann II GM, Hillman,EMC. "Direct, intraoperative observation of ~0.1 Hz hemodynamic oscillations in awake human cortex: Implications for fMRI." NeuroImage, 87, 323-331, (2014).
We have performed several studies looking at the post-natal development of neurovascular coupling in mice. This project is based on a range of studies in human neonates that have reported both positive and inverted fMRI BOLD and near infrared spectroscopy responses.
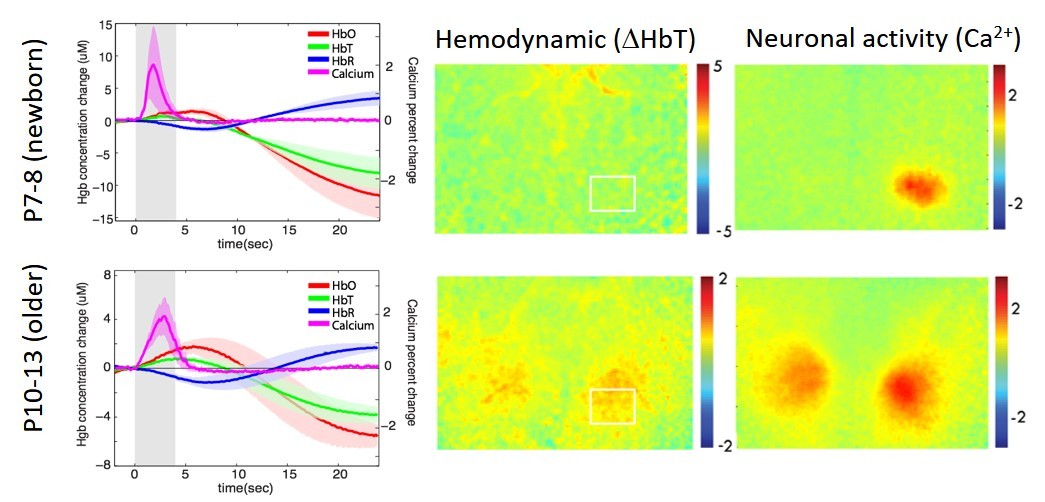
Our glioma project combines findings in human studies as described above, with studies of animal models of glioma developed by Dr Peter Canoll. Check out our latest Biorxiv preprint here.
Kozberg MG, Ma Y, Kim SH, A. SM, and Hillman EMC, "Rapid postnatal expansion of neural networks occurs in an environment of altered neurovascular and neurometabolic coupling". J Neurosci, , 36(25): 6704-6717 (2016).
Hillman, EMC. & Kozberg, MG. "What secrets can functional MRI reveal about the developing infant brain?" Imaging in Medicine 5, 203-206, (2013).
Kozberg M and Hillman EMC, "Chapter 10 - Neurovascular coupling and energy metabolism in the developing brain", in Progress in Brain Research, H.H. Kazuto Masamoto and Y. Katsuya, Editors. Elsevier. p. 213-242. (2016)
Kozberg MG, Hillman EMC, "Neurovascular coupling develops alongside neural circuits in the postnatal brain", Neurogenesis, 3(1): p. e1244439 (2016).
Kozberg M, Chen BR, De Leo SE, Bouchard MB, Hillman EMC, "Resolving the transition from negative to positive BOLD in the developing brain", PNAS, 110(11):4380-5 (2013).
Hillman EMC, "Out for Blood", Scientific American MIND, July / Aug (2014).
Hillman EMC, "Coupling Mechanism and Significance of the BOLD Signal: A Status Report", Annual Reviews, Neuroscience, 37, (in press) (2014).
Chen BR2, Kozberg MG2, Bouchard MB2, Shaik MA2, Hillman EMC, "A critical role for the vascular endothelium in functional neurovascular coupling in the brain", JAHA, 3: e000787, (2014).
Rayshubskiy A, Wojtasiewicz TJ, Mikell CB, Bouchard MB, Timerman D, Youngerman BE, McGovern RA, Otten ML, Canoll, PD, McKhann II GM, Hillman,EMC. "Direct, intraoperative observation of ~0.1 Hz hemodynamic oscillations in awake human cortex: Implications for fMRI." NeuroImage, 87, 323-331, (2014).
Hillman, E. M. C. & Kozberg, M. G. "What secrets can functional MRI reveal about the developing infant brain?" Imaging in Medicine 5, 203-206, (2013).
Kozberg M, Chen BR, De Leo SE, Bouchard MB, Hillman EMC, "Resolving the transition from negative to positive BOLD in the developing brain", PNAS, 110(11):4380-5 (2013).
McCaslin AFH, Chen BR, Radosevich AJ, Cauli B, Hillman EMC, "In-vivo 3D morphology of astrocyte-vasculature interactions in the somatosensory cortex: implications for neurovascular coupling", J Cereb
Blood Flow Metab, 31, 795-806 (2011). (Feature Article and Cover)
Chen BR, Bouchard MB, McCaslin AFH, Burgess SA, Hillman EMC, "High-speed vascular dynamics of the hemodynamic response", Neuroimage, 54 (2), 1021-1030 (2011).
Taber KH, Hillman EMC, Hurley RA, "Optical Imaging: A New Window to the Adult Brain", Neuropsychiatry Clin Neurosci. 22 (4), 357-360 (2010). (cover)
Tian P, Teng IC, May LD, Kurz R, Lu K, Scadeng M, Hillman EMC, De Crespigny AJ, D'Arceuil HE, Mandeville JB, Marota JJA, Rosen BR, Liu TT, Boas DA, Buxton R, Dale AM, Devor A, "Cortical depth-specific microvascular dilation underlies laminar differences in BOLD fMRI response", PNAS, 107 (34), 15246-15251 (2010).
Sirotin YB, Hillman EMC, Bordier C, Das A. "Spatiotemporal precision and hemodynamic mechanism of optical point-spreads in alert primates", PNAS 106, 18390-18395, (2009).
Bouchard MB*, Chen BR*, Burgess SA, Hillman EMC, "Ultra-fast multispectral optical imaging of cortical oxygenation, blood flow and intracellular calcium dynamics", Optics Express, 17 (18), 15670-15678, (2009).* Equal contribution first authors.**Also selected to appear in the Virtual Journal of Biomedical Optics, 4 (10), 15670.
Karagiannis A, Gallopin T, David C, Battaglia D, Geoffroy H, Rossier J, Hillman E.M.C, Staiger J, Cauli B. “Classification of NPY-expressing neocortical interneurons", J. Neurosci, 29(11):3642-3659 (2009).
Devor A, Hillman E. M. C, Tian P, Waeber C, Teng I. C, Ruvinskaya S, Shalinsky M, Zhu H, Haslinger R, Narayanan S, Ulbert I, Dunn A. K, Lo E, Rosen B. R, Dale A. M, Kleinfeld D, Boas D. A, “Stimulus-induced changes in blood flow and 2-deoxyglucose uptake dissociate in ipsilateral somatosensory cortex", J. Neurosci, 28: 14347-14357 (2008).
Hillman E. M. C, “Optical Brain Imaging In-vivo: Techniques and Applications from Animal to Man” [Invited Review] J Biomed Opt, 12(5), 051402 (2007). *Also selected to appear in the Virtual Journal of Biological Physics Research
Hillman E. M. C, Devor A, Bouchard M. B, Dunn A. K, Krauss GW, Skoch J, Bacskai J, Dale A. M, Boas D. A. “Depth-resolved Optical Imaging and Microscopy of Vascular Compartment Dynamics During Somatosensory Stimulation”, NeuroImage, 35(1): 89-104 (2007) Supplementary Data
Devor A, Tian P, Nishimura N, Teng IC, Hillman E. M. C, Narayanan S. N, Ulbert I, Boas D. A, Kleinfeld D, Dale A. M. “Suppressed neuronal activity and concurrent arteriolar vasoconstriction may explain negative BOLD”, J Neurosci, 27(16): 4452-4459. (2007) Supplementary Data